Water-Distribution System Modeling
as a Tool to Assist Epidemiologic
Investigations at Dover Township, New Jersey
Morris L. Maslia, M.
Jason B. Sautner, Mustafa M. Aral, Juan J. Reyes, John E. Abraham,
and Robert C. Williams
ABSTRACT
Toms River Areal View
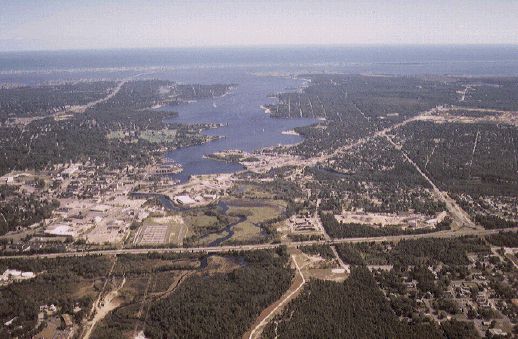
An epidemiologic
study of childhood leukemia and central nervous system cancers that
occurred in the period 1979 through 1996 in Dover Township, New
Jersey, is being conducted. Because groundwater contamination has
been documented historically in public- and private-supply wells,
there is the possibility of exposure through this pathway. The Dover
Township area has been primarily served by public water supply that
relies solely on groundwater; therefore, a protocol has been
developed for using a water-distribution model such as EPANET as a
tool to assist the exposure assessment component of epidemiologic
investigation. The model is being used to investigate the question
of human exposure to groundwater contaminants. Because of the
unavailability of historical data, the model was calibrated to the
present-day (1998) water-distribution system characteristics.
Pressure data were gathered simultaneously at 25 hydrants throughout
the distribution system using continuous recording pressure data
loggers during 48-hour tests in March and August 1998. Data for
storage tank water-levels, system demand, and pump and well status
(on/off) were also obtained. Field-data gathering procedures,
calibration results and water-quality simulation using a naturally
occurring element (barium), as well as an analysis indicating the
percent of water originating from points of entry to the
water-distribution system for 1998 conditions are presented.
On Toms River
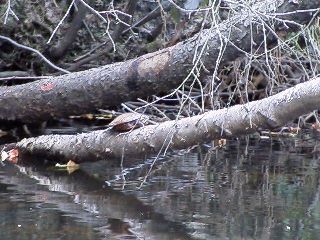
INTRODUCTION
In January 1997, the
Agency for Toxic Substances and Disease Registry (ATSDR) was
requested to assist with a case-control epidemiologic investigation,
being conducted by the New Jersey Department of Health and Senior
Services (NJDHSS), of childhood leukemia and central nervous system
cancers that occurred in the period 1979 through 1996
(Berry and Haltmeier, 1997) in the Dover Township area (Figure
1). Epidemiologic research generally employs non-experimental
(observational) studies in which exposure is not assigned or
controlled by the investigator, owing to ethical issues associated
with experimental studies. There are two types of observational
study designs that are most commonly used in epidemiologic
investigations: cohort and case-control studies. In a case-control
study, a population is delineated and cases of disease arising in
that population over a specified time period are identified. The
exposure experiences of the case group are compared to the exposure
experiences of a sample of the non-diseased persons in the
population from which the cases arose
(Rothman and Greenland, 1998). A case-control design is an
efficient method for studying risk factors for rare diseases such as
childhood cancers. For the case-control study being conducted by the
NJDHSS, environmental exposures that are more common in cases (cases
are children diagnosed with certain cancers) may be considered as
possible risk factors for disease (such as childhood leukemia and
brain and central nervous system cancers). One risk factor being
considered by ATSDR and NJDHSS is the potential for exposure to
contaminated potable water.
There have been
previous investigations trying to relate water-supply contamination
with health effects.
Lagakos et al. (1986) describe an association between exposure
to trichloroethylene-contaminated drinking water and increased
prevalences of stillbirths and central nervous system defects, oral
defects, and chromosomal defects. To investigate the potential
reproductive health effects of long-term, low-dose exposure to
waterborne chloroform,
Kramer et al. (1992) conducted population-based case control
analyses to study the association of trihalomethanes with low
birth-weight, prematurity, and intrauterine growth retardation using
state of Iowa birth certificate data.
Bove et al. (1995) used environmental and birth outcome
databases for a four-county area in northern New Jersey to study the
effects of public drinking water contamination on birth outcomes.
Toms River Marsh Area
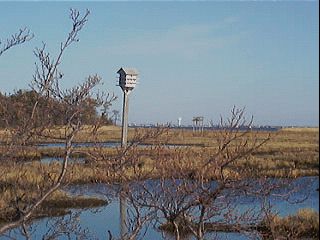
Ideally, to conduct
their investigation, the NJDHSS would have liked for ATSDR to
establish the concentration of water at the tap that a household
might have received. However, this task was not possible given the
paucity of contaminant-specific concentration data during the
desired study time frame. As an alternative, scientists from the
NJDHSS would like information on the percentage of water that a
person might have received during the study period from points of
entry to the water-distribution system serving the residents of the
Dover Township area (Figure
1). This would allow the investigators to assess the association
of the occurrence of childhood cancer with exposure to each of the
sources of potable water entering the distribution system, including
ones known to be historically contaminated.
EXPOSURE ASSESSMENT APPROACH
Groundwater
contamination of water-supply wells in Dover Township has generally
consisted of volatile organic compounds (VOCs) such as
trichloroethylene (TCE) and semi-volatile organic compounds (SVOCs)
such as styrene-acrylonitrile (SAN) trimer
(NJDHSS, 1999c). The reader is referred to the following reports
for a description and analysis of the contamination of groundwater
resources:
ATSDR (1988, 1989), Malcolm Pirnie, Inc. (1992), Pinder, et al.
(1992), and NJDHSS (1999a, b, c). Groundwater is the source of
potable water in the area and approximately 85 percent of the
current Dover Township area residents are served by a public-supply
system. Therefore, there exists the possibility of human exposure to
these contaminants through the groundwater pathway and an analysis
of the potential for distribution of contaminants through the
water-distribution system was deemed necessary.
In a distribution
system such as the one serving the residents of the Dover Township
area, not all public-supply wells may become contaminated.
Furthermore, some supply wells affect certain areas more than other
wells, and disease cases may have no relationship with contaminant
sources. Therefore, we will be using the concept of "proportionate
contribution" to assess the contribution of water from various
points of entry of the distribution system to subject residences.
Because the focus of the epidemiologic investigation is on children,
exposure at residential locations is deemed as the most important
exposure source to investigate, although other exposure sources may
be present. Based on residence histories, reconstruction of
historical water-distribution system characteristics can be used to
estimate exposure to specific water sources by determining the
percent of water study subjects may have received from each of the
points of entry to the water-distribution system.
It is important to
note that the analysis of the water-distribution that we have
undertaken, the proportionate contribution of different water
sources to the supply for cases and controls, is but merely one
component of an overall exposure assessment being conducted by the
NJDHSS to address issues of concern to the community. Other aspects
that are being addressed include obtaining valid residential
histories, estimating consumption patterns, conducting a study based
on data gathered from birth certificates, and the possibility of
exposure to contaminants from other environmental media such as air
and soil.
To address a
particular issue of concern to the community, assist the NJDHSS, and
reconstruct historical water-distribution system characteristics, we
have chosen to use a water-distribution system model. The use of
water-distribution system modeling for estimating exposure has been
described in the literature by several investigators.
Murphy (1986) calculated exposure to TCE from Wells G and H in
Woburn, Massachusetts by using a water-distribution model to assess
various pumping and water-use configuration patterns during each
month that the wells were in operation.
Clark et al. (1991) and Geldreich et al. (1992) used extended
period simulation hydraulic and dynamic water-quality models to
investigate the distribution of occurrences of illness due to
waterborne contaminants (escherichia coli sterotype 0157:H7)
found in the Cabool, Missouri, distribution system.
Clark et al. (1996a, b) used the EPANET water-distribution
system model to develop several scenarios to explain possible
pathogen transport of waterborne Salmonella typhimurium
outbreak in the Gideon, Missouri, municipal water system.
Aral et al. (1996) and Aral and Maslia (1997) used the EPANET
water-distribution system model in conjunction with a geographic
information system (GIS) to simulate four exposure scenarios for the
Southington, Connecticut, water-supply system that used groundwater
contaminated with VOCs during the 1970s. For our evaluation, the
EPANET water-distribution model, integrated with spatial analysis
technologies, is being used to model the water-distribution system
serving the residents of the Dover Township area.
In this paper, we
present three aspects of the exposure assessment effort that will
use a model for historical reconstruction of the hydraulic
characteristics of the water-distribution system. These aspects are:
(1) the field-data gathering procedures, (2) model calibration
results and water-quality simulation, and (3) simulations that have
been conducted on the present-day system to estimate the percent of
water originating from each of eight points of entry to various
locations throughout the water-distribution system.
Toms River Hydrant
Testing
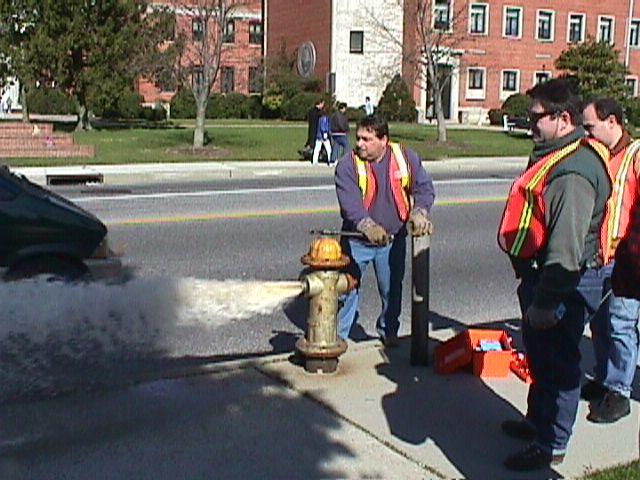
DESCRIPTION OF THE WATER-DISTRIBUTION SYSTEM
The
water-distribution system being investigated has been operating
since 1897. It serves the residents of Dover Township, New Jersey,
including the Toms River area, and communities outside of Dover
Township including the borough of South Toms River and a portion of
Berkeley Township (Figure
1). At the end of 1997, the water-distribution system served a
population of 92,160 persons that consisted of 44,510 customers. The
distribution system consists of 785.7 km (488.2 mi) of mains,
ranging from 40.6 to 5.1 cm (16 to 2 in.) in diameter, three
elevated and six ground-level storage tanks with a total rated
storage volume of 2.78x107 L (7.35 Mgal), 23 groundwater
extraction wells in eight well fields with a combined rated capacity
of 1.02x108 L/d (27 Mgal/d), and 17 high service or
booster pumps
(Board of Public Utilities, 1997). Water from seven of these
wells is pumped directly into the distribution system (e.g., wells
20, 31), whereas the remaining 16 wells are used to fill storage
tanks (e.g., well 40 and the Windsor ground-level storage tank) and
then high service or booster pumps are used to supply the
distribution system with water from the storage tanks (Figure
1).
Elevated Storage Tank
Demand for water in
the Dover Township area is characterized by two typical demand
patterns. A winter-time demand pattern, typical of data collected in
March 1998 (Figure
2A), generally occurs from October through mid-May of the year.
In March 1998, based on field observations
(Sautner and Maslia, 1998), demand was equal to 2.9x107
L/d (7.6 Mgal/d). Peak-demand conditions, typical of field data
collected in August 1998 (Figure
2B) and equal to 6.1x107 L/d (16.1 Mgal/d), generally
occur during the summer season from the end of May (Memorial Day)
through September of the year
(Maslia and Sautner, 1998b). Thus, the average annual demand
based on 1998 field data is approximately 4.5x107 L/d (12
Mgal/d).
COLLECTION
OF FIELD DATA
There were primarily
two reasons for developing a protocol for a synoptic, system-wide
collection of hydraulic and operational data. First, to understand
the present-day distribution of water from points of entry (sources)
to locations throughout the distribution system, a calibrated model
of the distribution system needed to be developed; however, a
database of spatially and temporally varying data by which to
characterize the distribution system was not available. Second, to
reconstruct historical characteristics of the water-distribution
system, a synoptic, system-wide characterization of the
water-distribution system, based on measured data was required.
Neither present-day or historical system-wide pressure measurements
were available for the water-distribution system being investigated.
Hence, investigators decided to obtain present-day measurements in
order to accurately characterize the water-distribution system.
ATSDR investigators,
in coordination with the NJDHSS and the water utility operators,
developed a protocol to collect pressure data and operational
information during winter- and peak-demand periods of the year–March
and August 1998, respectively. Details of the protocol are provided
in
Maslia and Sautner (1998a) and are briefly described below.
Hydrant Selection
Twenty-five hydrants
(out of a system total in 1997 of 2,127) were initially selected as
test hydrants (designated as H-1, H-2, etc.) on which continuous
pressure recording equipment would be installed. The number and
locations of the test hydrants (H-1, H-2, etc., shown in
Figure 1) were selected based on the following considerations:
-
Initial
simulations conducted with EPANET indicated areas of
questionable high pressure (exceeding 862 kPa (125 psi))
within the water-distribution system. The data used as input
for the simulations were supplied by the water-utility.
According to water-utility staff, the data represented an
equivalent hydraulic network of 15.2 cm (6 in.) and larger
diameter pipelines for 1993 conditions
(Flegal, 1997). Therefore, hydrant locations were
selected to assist in determining the actual pressures that
exist in these areas.
-
Hydrant
locations were also selected to provide as complete as
possible a system-wide coverage so that effects from storage
tank and pump operations could be characterized by pressure
changes at these hydrants.
-
ATSDR used
additional hydrants for quality assurance purposes in the
event that data collection devices failed to operate
properly, or that hydrants became inoperable or unusable
during the test.
-
ATSDR
responds to and tries to accommodate stakeholder input when
conducting site activities. In the case of modeling the
water-distribution system serving Dover Township, area
residents wanted assurances that a sufficient number of
measuring locations would be available to accurately
characterize the distribution system. Because ATSDR
scientists determined that additional data-collection
locations would not abrogate the scientific merits of the
field test, additional monitoring locations requested by
area residents were also included.
As part of a quality
assurance program, 25 hydrants were selected as alternate test
hydrants (designated as AH-1, AH-2, etc.) in the event that any of
the original 25 test hydrants could not be used to monitor pressure
during the test periods. During the installation of the data
gathering equipment on the hydrants, it was determined that 5 of the
designated test hydrants (H-5, H-6, H-14, H-19, and H-22) were not
suitable for use as measuring points. Therefore, the associated
alternate hydrants (AH-5, AH-6, AH-14, AH-19, and AH-22) were used.
The final set of test hydrants used for both the March and August
1998 tests is shown in
Figure 1. These 25 hydrants are connected to network pipelines
that: (1) were installed between 1963 and 1996, (2) are constructed
of asbestos cement (14 hydrants), plastic (PVC, 10 hydrants), and
ductile iron (1 hydrant) materials, and (3) range in diameter size
from 30.5 to 15.2 cm (12 to 6 in.).
Data Recording Equipment
RADCOM Lolog LL(TM)
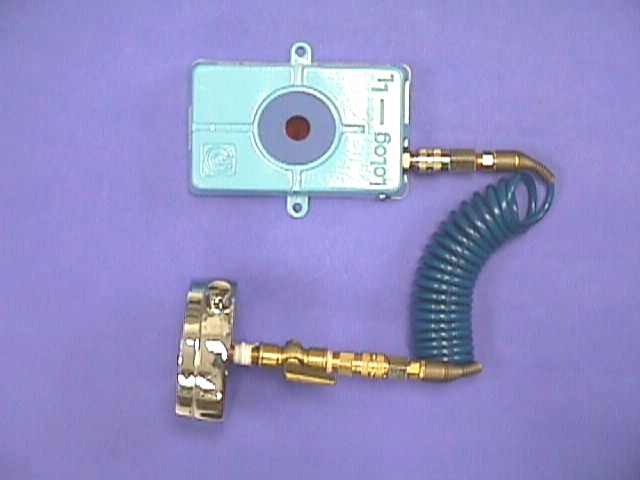
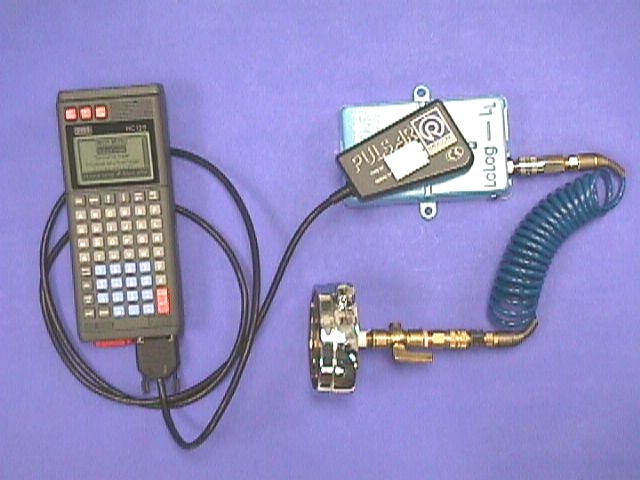
Pressure Gage
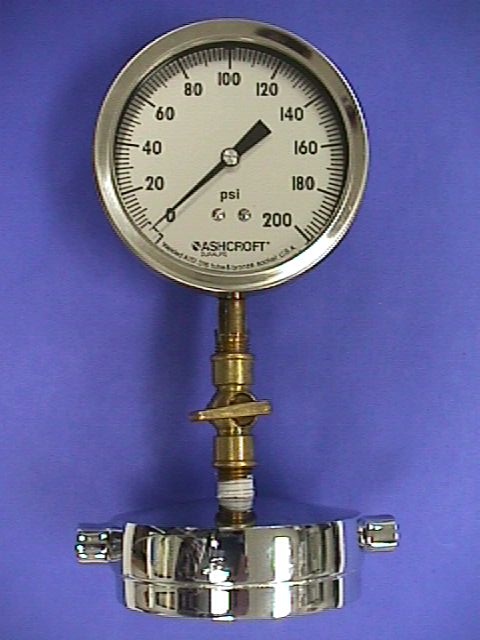
Each test hydrant was
equipped with a RADCOM Lolog LL™ continuous pressure data logger
that sampled pressures at 1-minute intervals. The intent of the test
was to collect time-series pressure data over a 48-hour test period.
At the conclusion of the test, data from each logger was downloaded
to a Psion HC-120™ hand-held computer for storage and post-test
analysis.
Test Protocol
A test protocol was
developed by ATSDR to insure that successful data-gathering would
occur during field-test activities and to establish quality
assurance procedures that would be followed for each hydrant to be
monitored
(Maslia and Sautner, 1998a). Prior to conducting the tests, the
loggers were factory calibrated to 1034 kPa (150 psi). The following
procedure was used to install and quality-assure the installation on
each of the 25 hydrants for the March and August 1998 field tests:
-
A hydrant was
opened and flushed to remove debris; when clear running
water was observed, the hydrant was shut off.
-
A hydrant
adapter kit, brass shut-off valve, and pressure gauge were
installed on the hydrant.
-
The hydrant
was reopened, and a pressure reading was obtained from the
pressure gauge and recorded in a log book. At this point, a
check was made for any leaks occurring around the pressure
gauge connection and the hydrant adapter kit.
-
The pressure
gauge was removed and the RADCOM Lolog LL pressure data
logger was now attached to the hydrant and hydrant adapter
kit, and a check was made for any leaks occurring around the
pressure data-logger connection and the hydrant adapter kit.
A pressure reading was obtained from the logger and compared
with the gauge pressure recorded earlier.
-
ATSDR staff
in the water utility’s operations control room recorded the
operational history of wells and booster pumps for the
duration of the tests. They also manually recorded
instantaneous system production data and storage tank water
levels being recorded electronically by the water utility’s
supervisory control and data acquisition (SCADA) system.
Throughout the tests,
ATSDR and NJDHSS staff periodically checked each test hydrant and
pressure data logger to assure that the logger was functioning
properly and that water leaks, potentially affecting the pressure
measurements, had not developed. After the test was concluded, the
loggers were removed by reversing the process described in steps 1-4
above, and the data was downloaded to and stored in the hand-held
Psion HC-120™ computer. Analysis of pressure data from both tests
indicate that throughout the water-distribution system pressures
generally range from a low of 276 kPa (40 psi) to a maximum of
slightly more than 689 kPa (100 psi). Details of the tests are
provided in reports by
Sautner and Maslia (1998) and
Maslia and Sautner (1998b).
WATER-DISTRIBUTION SYSTEM MODEL DEVELOPMENT
Historical Background
Using mathematical
models to analyze flow in water-distribution system networks has
been in existence for more than 60 years since it was proposed by
Cross (1936) in the 1930s. Hydraulic models can be used to
analyze systems where demand and operating conditions are static or
are time varying. The former is a ‘steady-state’ model, and the
latter is referred to as an ‘extended period simulation’ (EPS)
model.
Modeling the spatial
distribution of water quality in pipelines first began with a
steady-state modeling approach suggested by
Wood (1980) who studied slurry flow. The representation of
temporally varying conditions for contaminant movement in a
distribution system or ‘dynamic’ water-quality models began to be
used in the mid-1980s. With the widespread use and relatively low
cost of personal computers and desktop workstations during the
mid-1980s and 1990s, there are now many models, both proprietary and
public domain, that can be used to conduct hydraulic and
water-quality analyses. The reader is referred to
Rossman (1999) and
Clark (1999) for a thorough discussion on the development and
evolution of hydraulic and water-quality models.
Hydraulic and Water-Quality Simulation Model
Hydraulic modeling of
water-distribution systems can be conducted by solving mathematical
equations that describe the pipe network of the distribution system.
Extended period simulation represents a succession of steady-state
solutions as demands and tank levels change over fixed points in
time. For each point in time, at network nodes (junctions), an
equation for conservation of mass is solved. At each network pipe
(link), an equation relating head loss and flow is solved. The
mathematical equations for the development of the hydraulic
simulation model and the numerical methods used to solve the
equations are referenced in the published literature, and because of
space limitations, will not be given here. For details, the reader
should refer to
Rossman (1994), Rossman et al. (1994), Todini and Pilati (1987), and
Salgado et al. (1988).
The fate of a
dissolved substance flowing through a distribution network over time
is tracked by EPANET’s dynamic water quality simulator. To model
water quality of a distribution system, EPANET uses flow information
computed from the hydraulic simulation as input to the water-quality
model. The water quality model uses the computed flows to solve the
equation for conservation of mass for a substance within each link.
The equations used to describe the fate and transport of a substance
flowing through a distribution network by EPANET and the numerical
method used to solve the equations are described in
Rossman (1994) and Rossman et al. (1993, 1994).
The identification of
the source of delivered water in a distribution system has become a
necessity when attempting to determine the location of a source that
may supply water that exceeds a given level of a chemical or
biological constituent.
Wood and Ormsbee (1989) developed an explicit method to
calculate the percentage of flow originating at various source
points at a specific location in a distribution system. EPANET also
has the ability to track the percent of water reaching any point in
the distribution network over time from a specified location
(source) in the network. Given the multiple number of points of
entry to the water-distribution system serving the residents of
Dover Township, the ability to track the percent of water
originating from a point of entry becomes a very useful analysis
tool for our investigation.
HYDRAULIC MODEL CALIBRATION
Overview
With a computer model
of the water-distribution system, we are trying to reproduce the
behavior of a real hydraulic system as closely as possible in terms
of spatial and temporal characteristics. The collection of field
data provides an opportunity to understand the operation of the real
system at a specified number of locations and times. Such efforts
are consistent with the American Water Works Association Engineering
Computer Applications Committee who find that "true model
calibration is achieved by adjusting whatever parameter values need
adjusting until a reasonable agreement is achieved between
model-predicted behavior and actual field behavior
(AWWA Engineering Computer Applications Committee, 1999)." Once
a model is considered to be calibrated, it can then be used to,
among other purposes, estimate hydraulic characteristics of the real
system at locations where measured data are unavailable or unknown,
spatially and temporally.
According to the
AWWA Engineering Computer Applications Committee (1999) there
are 10 sources for possible error that could cause poor agreement
between simulated model values and measured field values. These
sources of error provide a potential list of parameter values that
can be adjusted during the model calibration process and are: (1)
errors in input data (measurement and typographical), (2) unknown
pipe roughness values (i.e., Hazen-Williams "C-Factors"), (3)
effects of system demands (distributing consumption along a pipe to
a single node), (4) errors in data derived from network maps, (5)
node elevation errors, (6) errors introduced by time variance of
parameter values such as tank water levels and pressures, (7) errors
introduced by a skeletal representation of the network as opposed to
modeling all small-diameter pipes, (8) errors introduced by
geometric anomalies or partially closed valves, (9) outdated or
unknown pump-characteristic curves, and (10) poorly calibrated
measuring equipment including data loggers, tank water-level
monitors, and SCADA systems. Owing to brevity and space limitations,
it is not feasible, in this paper, to discuss each of these sources
of error with respect to the data obtained for the distribution
system under consideration. However, after careful review of the
data and the possible sources of error, investigators narrowed the
list to three parameters that are believed provide the greatest
source for error for our situation and therefore, subjected to
possible adjustment during the calibration process. The parameters
are: (1) pipe roughness values (Hazen-Williams "C-Factors"), (2)
system demand factors, and (3) pump-characteristic curves (head
versus flow values). A detailed discussion of values assigned to
these parameters and adjustments made during the model calibration
process is provided below in the section on "Calibration Parameters
."
Data Sources and Calibration Methodology
Physical
characteristics of the distribution system (pipe, pump, storage
tank, and well data) were obtained from databases supplied by the
water utility
(Flegal, 1997). Sources for the data and model parameter values
are listed in
Table 1. Based on the information supplied by water utility,
ATSDR developed a model network consisting of 16,071 pipeline
segments (links) and 14,987 junctions (nodes).
Table 2 describes the material type for pipes composing the
network as of the end of 1997, and as shown, most of the network is
composed of asbestos cement and plastic (PVC) pipes. Because the
goal of our investigation is to conduct a population-based
assessment, geo-spatial location information for network facilities
was required. Therefore, geographic coordinates for the network
nodes were determined by using global positioning system (GPS)
equipment to obtain locations of the 50 test and alternate hydrants,
wells, and storage tanks, in conjunction with
TIGER/Line™ files (1992) for the Dover Township area. In
addition to spatial coordinates, land surface elevation values for
the measuring points (hydrants) were also obtained by using GPS
equipment. Elevation values for network nodes were obtained from
7.5-minute U.S. Geological Survey Digital Elevation Model
quadrangles for the Dover Township area and assigned to network
nodes by using GIS software.
The model was
calibrated to the hydraulic and operational data collected during
the March 1998 test and then confirmed against data collected during
the August 1998 test. The model was run as an EPS using one-hour
hydraulic time steps and demand-pattern factors derived from the
control-room data collected during the tests (Figure
2a and
Figure 2b). Consumption data required by the model at nodal
locations throughout the distribution service area were based on
metered billing records collected quarterly by the water utility,
from October 1997 through April 1998. The consumption data were
allocated by using an address-matching technique and GIS software to
locate the water meter address to the nearest model node.
In order to
accommodate the hourly-time step pattern of the EPS required by
EPANET, the one-minute sampling data that were collected during the
field tests were averaged to one-hour time periods. Hourly pressure
data, based on the one-minute sampling data for 5 of the 25 test
hydrants are shown in
Figure 3 for the March and August 1998 tests. Because of space
limitations, it is not possible to show data and results for all 25
test hydrants in this paper. However, it is important to note that
the model calibration analyses and results are based on simulations
from all 25 hydrants. Therefore, further discussion of simulation
results will focus on the 5 hydrants that represent the southwest
(test hydrant H-1), south-central (test hydrant H-11), central (test
hydrant H-17), northwest (test hydrant H-20), and northeast (test
hydrant AH-22) portions of the study area (Figure
1).
To simulate
groundwater wells pumping directly into the distribution system or
into storage tanks, well-flow data from the SCADA system was
recorded by ATSDR staff during the tests. For model calibration
purposes, flow from multiple wells in a well field to a tank, such
as Parkway well field or Holly Plant well field (Figure
1), was combined and assumed to be one well. The well (or
combined wells for a well field) was associated with a model
junction and a negative base demand was assigned to the junction,
indicating inflow to the network or to a tank. Because EPANET allows
one to assign a different demand pattern to each junction in the
model, each well or well field was assigned a demand pattern based
on the recorded SCADA-system data. These demand patterns were used
to simulate varying flow rates and the on/off cycling of the wells
during the test.
Comparisons of
measured pressure with simulated pressure for the March and August
1998 tests at the 5 test hydrants are presented in
Figure 3. Comparisons between measured and simulated hydraulic
heads are presented in
Figures 4A and
4B. (Measured hydraulic heads were derived from measured water
levels in the tanks and the elevation of the bottom of the tanks.)
Comparison of measured and simulated pump flows are presented in
Figure 5. Described below are the model parameters that were
adjusted for the calibration process and analysis of the results of
the March and August 1998 simulations.
Calibration Parameters
Three model
parameters were considered for variation with respect to model
calibration: (1) pipe roughness (Hazen-Williams "C-Factor"), (2)
system demand factors, and (3) pump-characteristic curves (head
versus flow values). Discussions with the water utility staff
revealed that the network pipes had very little scaling or
deposition, and inspections have shown very little debris.
Additionally, as shown in
Table 2, more than one-third of the pipes (in quantity and
lengthwise) are made of PVC where the variation in "C" is
negligible. Therefore, initial estimates for "C", obtained from
published tabular values
(Rossman, 1994) and listed in
Table 2 for every pipe material type, were not varied during the
calibration process. Subsequent to model calibration, a sensitivity
analysis confirmed that for our investigation, variation in
"C-Factor" has an insignificant influence on system pressures and
flow directions.
Initial estimates of
system demand factors were obtained by ATSDR staff recording
production data during the tests from the control-room SCADA-system
output. In EPANET, the system demand factors are used to distribute
the average daily nodal consumption values over hourly time steps of
an EPS. Therefore, for the March and August 1998 tests, the system
demand factors distributed the average nodal consumption values over
the 48 hourly time steps of the EPS that were used to model the
field tests. During the calibration process, the individual hourly
factors were modified. The factoied by a trial and
error method using the generalized diurnal pattern shown in
Figures 2a and
2b as guidance for adjusting the factors (e.g., peak demand
occurring at approximately 0800 and 1900 hours; low demand occurring
at approximately 0300 and 1600 hours). Factors for the August test
were modified from factors for the March test because the conditions
of each test represented different demand conditions–winter-time
demand for the March test and peak demand for the August test. It is
important to note, however, that although individual hourly factors
were modified, the total system-wide demand, 2.9x107L/d
(7.6 Mgal/d) for March and 6.1x107 L/d (16.1 Mgal/d) for
August was not modified during the calibration process. The
calibrated values for the hourly demand factors for the March and
August 1998 test are shown in
Figures 2a and
2b.
Consumption data are
needed as input for EPANET at nodal locations throughout the model
network that represents the distribution system service area. These
data were provided by the water utility and represented metered
billing records collected quarterly from October 1997 through April
1998. The consumption data were distributed to model nodes by using
an address-matching technique and GIS software to assign the water
meter address to the adjacent (and nearest) model node. If more than
one meter address was adjacent to a model node, then the consumption
for the node was the sum of all metered consumption adjacent to the
node. Because our model is composed of pipes as small as 5-cm
(2-in.) in diameter, the density of the model network is such
(14,987 nodes) that errors due to incorrect or inaccurate
distribution of consumption are minimal and insignificant.
Therefore, the spatial (nodal) distribution of consumption in terms
of an average daily value derived from records provided by the water
utility, was not modified during the calibration process. Rather, as
discussed above, the system demand factor pattern in EPANET was used
to distribute the average daily consumption value to an hourly time
step value.
A third model
parameter that was adjusted during the calibration process was the
high service and booster pump-characteristic curves. Initial
pump-characteristic curve data were provided to ATSDR by the water
utility
(Flegal, 1997), and these are listed in
Table 3. However, the source of the data (manufacturer’s data,
field testing, etc.) was unknown. Therefore, this was a key
parameter that could justifiably be modified during the calibration
process. Modifications were made to the original data so that system
operations, as observed during the two tests, could be duplicated as
closely as possible. The calibrated pump-characteristic curve data
are also listed in Table 3 along with the status of the pumps
(on/off) during the March and August 1998 tests. Out of the 11
system pumps listed in
Table 3, 3 pumps (Holly booster 2, Parkway booster 2, and Route
37) required little or no modification during the calibration
process. Two of the pumps (South Toms River booster 1 and 2) were
not operated during either the March or August 1998 test.
Calibration Statistics
In an effort to
assess the overall quality and reliability of the model calibration,
analyses based on calibration statistics were conducted. The
calibration statistic used for our analyses is the absolute mean
pressure difference, which for our investigation is defined as the
absolute difference between a measured pressure value (based on a
one-hour average of one-minute sampling data) and a simulated
pressure value. We will refer to this difference as "model error."
A comparison model
error by hydrant (or measuring point) location is presented in
Figure 6. The bars on the graph represent the mean of absolute
pressure difference and were computed by averaging the model error
for each hydrant over the 48-hour test period. The graph indicates
that for March 1998, the model error range is 9.6 to 54.4 kPa (1.4
to 7.9 psi), and for August 1998, the model error range is 20.0 to
45.5 kPa (2.9 to 6.6 psi). System-wide model error for March 1998 is
lower than for August 1998, and with the exception for hydrants H-2
and H-3, model error for March 1998 is less that 34.5 kPa (5 psi).
For August 1998, system-wide model error is also less than 34.5 kPa
(5 psi) except for hydrants H-4, AH-5, H-9, and H-13.
The reliability of
the calibrated model can be judged in terms of the frequency of
model error. The graph in
Figure 7 indicates that for the March 1998 simulation,
approximately 90 percent of the simulated values result in a model
error of 34.5 kPa (5 psi) or less. For the August 1998 simulation,
the data indicate that approximately 70 percent of the simulated
values result in a model error of about 34.5 kPa (5 psi) or less.
The largest model
errors occur in the South Toms River and Berkeley Township areas
(Figure 1), and overall, the March 1998 simulation, has a smaller
model error (Figure
6,
7). Primarily we attribute this characterization of model error
to the lack of metered consumption data availability specifically
during the test periods. As discussed above, metered consumption
data were available for the Dover Township area serviced by the
water-distribution system solely on a quarterly basis; thus, each
meter was read four times per year. However, all meters were not
read at the same time nor within a few days of each other. Thus, for
the March 1998 simulation, quarterly consumption data for October
1997 through April 1998 was available. This same data was used for
the August 1998 simulation because metered data representing
consumption conditions for August 14-16, 1998 or for the peak-demand
season were not available for model simulation and calibration. To
further reduce model error, therefore, it is our opinion that
metered consumption data (and consequently, nodal consumption data)
during the time of the test, and specifically, during the
peak-demand test, would be required.
Overall, we believe
the model errors for both March and August simulations are within
acceptable limits for our purpose and that the calibrated model is
an accurate characterization of the water-distribution system for
winter-demand and peak-demand conditions of 1998. In addition to the
analysis of model error, comparison of measured and simulated
pressures for the 5 hydrants presented in this paper (Figure
3), the comparison of measured and simulated hydraulic head at
ground-level and elevated storage tanks (Figure
4a and
4b), and comparison of measured and simulated booster pump flows
(Figure
5), support our assertion that the model presented herein is an
acceptable and reliable representation of water-distribution system
conditions existing during 1998.
WATER-QUALITY SIMULATION
To calibrate a model
for use in fate and transport simulations, field data pertaining to
system flows and the movement of a tracer should be obtained. At the
time that the pressure data were collected, however, it was not
possible to collect flow and water-quality data because of
institutional, operational, and budgetary constraints. ATSDR
investigators did obtain water-quality data from a one-time sampling
event that occurred on March 28, 1996
(NJDHSS, 1999c). We use these data to compare with results of a
water-quality simulation as further evidence that the model we have
developed of the water-distribution system is reasonably calibrated.
On March 28, 1996,
NJDHSS collected water samples from taps at 21 schools located
throughout Dover Township and serviced by the water utility (Figure
8). The samples were analyzed for, among other constituents, the
naturally occurring element, barium. Barium is assumed to be a
conservative substance. On April 4, 1996, NJDHSS also collected
water samples from 5 points of entry to the distribution system (the
assumed sources for the barium). These points of entry were (Figure
8): well 32 (South Toms River), well 20 (Indian Head), well 31
(Route 70), and the Parkway storage tank. An additional point of
entry was sampled on April 24, 1996, Holly Plant storage tank. This
point of entry was sampled at a later date than the other points of
entry because all of the wells supplying the tank (wells 21, 30, and
37) were off-line on the day the other points of entry were sampled
(April 4, 1996), but well 30 was on-line when the tap samples were
obtained at the 21 schools (March 28, 1996). The measured
concentration of barium at each of the points of entry is shown in
Figure 8.
To simulate the
spatial distribution of barium, information on the operating
schedule for pumps and wells (cycling on/of schedules) and on tank
levels for March 28, 1996 was obtained from the water-utility staff.
Additionally, data for the daily production for March 28 was also
obtained. The calibrated system demand factors were modified so that
the average daily production for March 28, 1996 (which was greater
than the calibration period of March 24, 1998) could be distributed
on an hourly basis. The concentration for barium measured at the
points of entry was used as the source concentration for the model
node associated with the point of entry. For the EPANET simulation,
the hydraulic time step was set at 1 hour and the water-quality time
step was set at 5 minutes. Initial conditions must be "flushed out"
of the distribution system prior to retrieving fate and transport
simulation results. Based on our model of the distribution system,
it takes approximately 1,000 hours (42 days) for the entire system
to reach a dynamic equilibrium condition (repeating pattern of tank
filling and emptying). Once dynamic equilibrium was reached, EPANET
was run for an additional 24 hours. The concentration at model nodes
corresponding to the 21 school locations is reported for 08:00
hours, the approximate time of the sample collections. Comparison of
measured and simulated barium concentrations (Figure
8) indicates a difference ranging from 0.2 to 12.4 mg/L which
results in a mean absolute difference of 13.6 percent with a range
of 0.6 to 25.6 percent. Thus, the fate and transport simulation of
barium provides further evidence that the model we have developed of
the water-distribution system serving the residents of Dover
Township is reasonably calibrated.
USE OF THE MODEL FOR THE EPIDEMIOLOGIC INVESTIGATION
The NJDHSS would have
liked for ATSDR to establish the historical concentration of tap
water at residences serviced by the public water supply. However,
this task is not possible given the paucity of concentration data
during the desired study time frame. Therefore, to assist the
epidemiologic case-control investigation, ATSDR will be providing
information on the percent of water study subjects may have received
from each point of entry to the water-distribution system–the
concept of "proportionate contribution." As an example, for the
present-day system (1998), this task can be accomplished by using
the trace analysis option within EPANET. For each point of entry to
the water-distribution system for a water source
(Table 4), a trace analysis was conducted. Once dynamic
equilibrium was reached, a 24-hour trace analysis simulation was
conducted by assigning a trace node to coincide with a point of
entry. Results of the trace analyses are obtained in terms of the
percent of water that any location of interest receives from the
trace node (water source) as an average over a 24-hour time period.
We chose the 5 hydrant locations previously discussed as points of
interest. Results of the trace analysis for March and August 1998,
representing winter-demand and peak-demand conditions, are presented
in
Table 4, in terms of the percent of water from the points of
entry to hydrants H1, H-11, H-17, H-20, and AH-22. A discussion of
the results of the trace analyses follows.
At the location
represented by test hydrant AH-22 (northeast area of Dover Township,
Figure 1), under winter-demand conditions (March 1998), the
Berkeley wells supply 7 percent of the water, the Route 70 (well 31)
supplies 26 percent of the water, and the Parkway well field
ground-level storage tank supplies 66 percent of the water. Under
peak-demand conditions (August 1998), the Berkeley wells supply less
than 1 percent of the water, Route 70 (well 31) and the Holly plant
ground-level storage tank supply about 1 percent of the water,
Brookside wells 15 and 43 supply about 8 percent of the water, and
the Parkway well field and Windsor ground-level storage tanks supply
82 and 6 percent of the water, respectively, to the area of test
hydrant AH-22. Thus, in terms of an exposure assessment on an
average day in 1998, a person residing in an area represented by
test hydrant AH-22 would receive more than 80 percent of their
potable water from the Parkway well field tank during summer time
(peak-demand) conditions, and about 66 percent of their potable
water from the Parkway well field tank during winter time
(winter-demand) conditions. To illustrate the spatial distribution
of the contribution of water from the Parkway well field tank during
winter-time and peak-demand conditions, results of the trace
analysis from the Parkway well field tank for March and August 1998
for are shown in
Figures 9 and
10, respectively. Comparison of these two analyses shows that
the amount of water contributed by the Parkway tank in August 1998
to the southeastern and eastern parts of the distribution system (Figure
10) is considerably reduced or eliminated when compared with the
March 1998 conditions (Figure
9). This is a result of well 40 and the Windsor ground-level
tank being in operation for the peak-demand conditions existing in
August 1998. As a consequence of this operation, the Windsor tank
meets the demand for water in the southeastern and eastern parts of
Dover Township serviced by the distribution system. The trace
analysis also shows the contribution of water from the Parkway well
field tank to the northern area of Dover Township serviced by the
distribution system increases from 50-75 percent in March (Figure
9) to 75-100 percent in August (Figure
10). Additionally, in the northern area of Dover Township
between the Holiday City ground-level tank and the North Dover
elevated tank, the Parkway ground-level tank does not supply any
water in March 1998 (Figure
9), but supplies between 10 and 50 percent of the water demand
in August 1998 (Figure
10).
To assist the
case-control epidemiologic investigation, therefore,
water-distribution system networks representing the location of
pipelines from 1962 through 1996 based on historical information
will be derived. Using historical information on system production,
hydraulic device location, and system operation (when available),
trace analyses will be conducted for three demand periods for each
year from 1962 through 1996. The demand periods are: (1) minimum
demand, (2) peak-demand, and (3) average demand. This will enable
ATSDR to provide information to epidemiologists and other health
scientists that can be used to relate study subject addresses to
areas historically served by the water-distribution system using
spatial analysis and address-matching techniques. Results of the
trace analyses will be provided in terms of "minimum day",
"peak-day", and "average-day" conditions for each year from 1962
through 1996. ATSDR modelers will be blinded to the status (case or
control) of study participants. Based on residence histories,
historical model trace simulation results will be used to estimate
exposure to specific water sources by determining the percent of
water, both cases and controls may have received from each of the
points of entry to the water-distribution system.
SUMMARY
An epidemiologic
study of childhood leukemia and central nervous system cancers that
occurred in the period 1979 through 1996 in Dover Township, New
Jersey, is being conducted. Because groundwater contamination has
been documented historically in public- and private-supply wells,
there is the possibility of exposure through this pathway. The Dover
Township area has been primarily served by public water supply that
relies solely on groundwater; therefore, a protocol has been
developed for using a water-distribution model (e.g., EPANET) as a
tool to assist the exposure assessment component of epidemiologic
investigation. To accomplish this, we will be using the concept of
"proportionate contribution" to assess the contribution of water
from various points of entry of the distribution system to subject
residences. In this paper, we have presented three aspects of the
overall exposure assessment effort that will use the model for
historical reconstruction of the proportionate contribution of
different water sources to the supply for cases and controls. These
aspects are: (1) the field-data gathering procedures, (2) model
calibration results and water-quality simulation, and (3)
simulations that have been conducted on the present-day system to
estimate the percent of water originating from points of entry to
various locations throughout the water-distribution system.
Acknowledgments
The authors would
like to acknowledge the following organizations for providing
information, assistance, personnel, and suggestions in developing
the protocol and conducting the field investigations described
above: ATSDR, NJDHSS, the New Jersey Department of Environmental
Protection, United Water Toms River, Inc., and the Multimedia
Environmental Simulations Laboratory at the Georgia Institute of
Technology. The authors would like to specifically acknowledge
Jerald A. Fagliano of the NJDHSS for suggestions and advice on the
epidemiologic aspects of this investigation, and Lewis Rossman of
the U.S. Environmental Protection Agency, National Risk Management
Laboratory, for assistance with and requested modifications to the
EPANET water-distribution system model. Their invaluable assistance
have made this effort possible.
Trademarks
The use of brand or
trade names is this paper is for identification purposes only and
does not constitute endorsement by the U.S. government or Georgia
Institute of Technology.